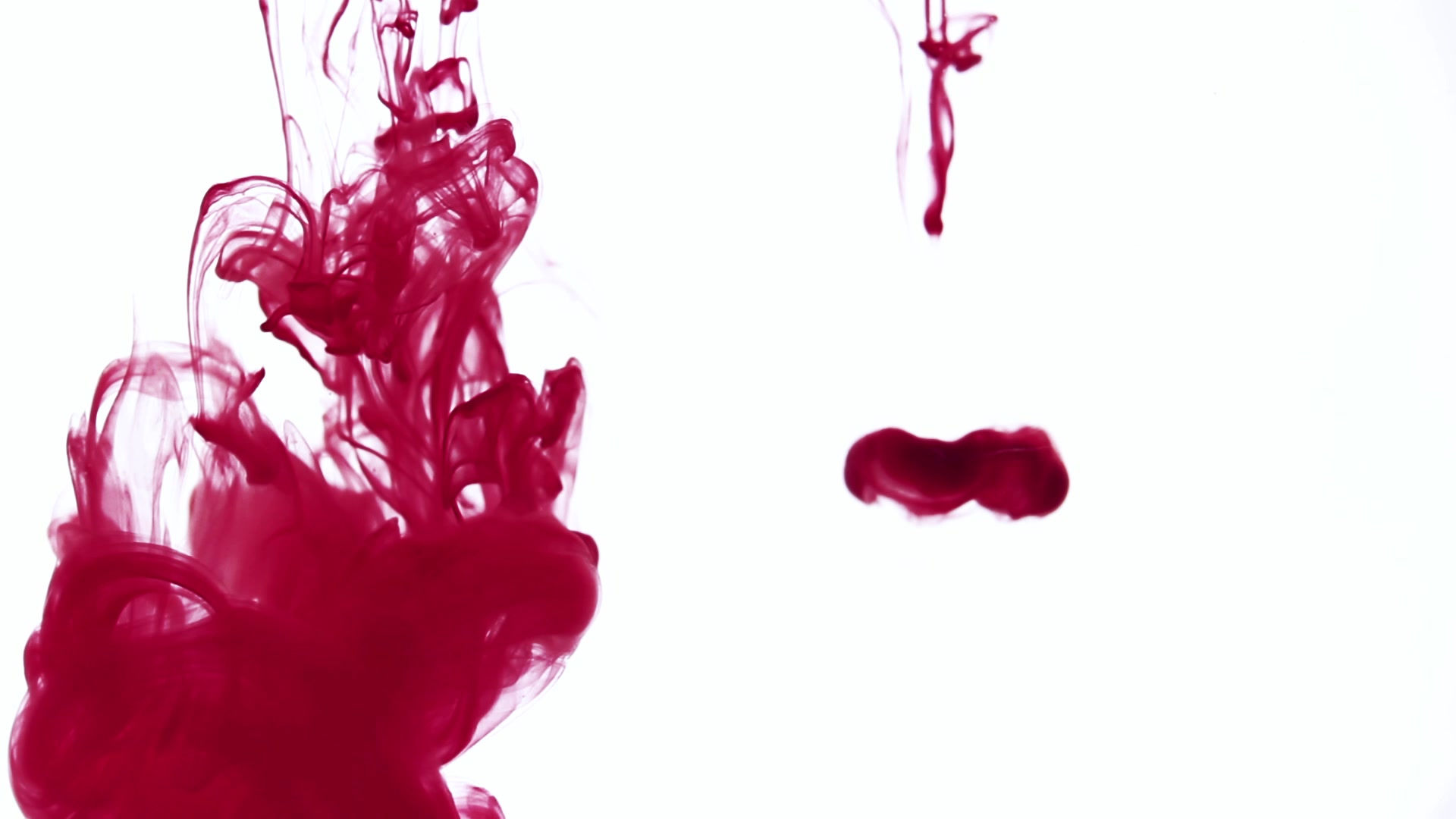
Kissimmee Homeschooling

Geography
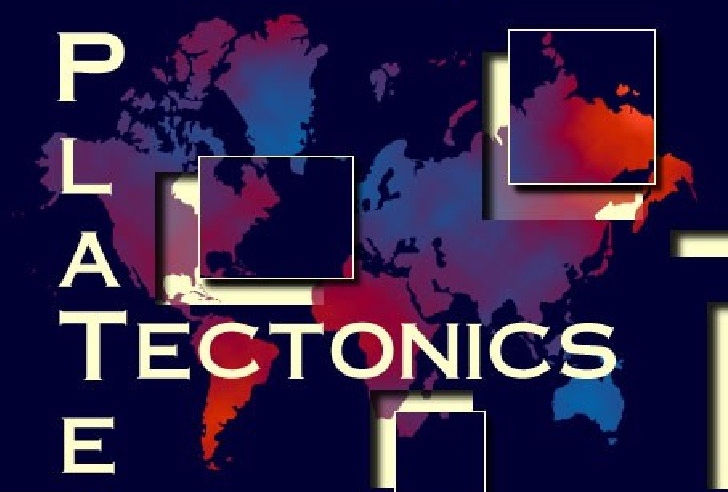
Plate Tectonics and Expanding Earth Theories
Continental drift is the movement of the Earth's continents relative to each other, thus appearing to "drift" across the ocean bed. The speculation that continents might have 'drifted' was first put forward by Abraham Ortelius in 1596. The concept was independently and more fully developed by Alfred Wegener in 1912, but his theory was rejected by some for lack of a mechanism (though this was supplied later byArthur Holmes) and others because of prior theoretical commitments. The idea of continental drift has been subsumed by the theory of plate tectonics, which explains how the continents move.
In 1858 Antonio Snider-Pellegrini created two maps demonstrating how America and Africa continents might have once fit together. Abraham Ortelius (Ortelius 1596), Theodor Christoph Lilienthal (1756), Alexander von Humboldt (1801 and 1845), Antonio Snider-Pellegrini (Snider-Pellegrini 1858), and others had noted earlier that the shapes of continents on opposite sides of the Atlantic Ocean (most notably, Africa and South America) seem to fit together. W. J. Kious described Ortelius' thoughts in this way: Abraham Ortelius in his work Thesaurus Geographicus ... suggested that the Americas were "torn away from Europe and Africa ... by earthquakes and floods" and went on to say: "The vestiges of the rupture reveal themselves, if someone brings forward a map of the world and considers carefully the coasts of the three [continents]."
From the deepest ocean trench to the tallest mountain, plate tectonics explains the features and movement of Earth's surface in the present and the past. Plate tectonics is the theory that Earth's outer shell is divided into several plates that glide over the mantle, the rocky inner layer above the core. The plates act like a hard and rigid shell compared to Earth's mantle. This strong outer layer is called the lithosphere.
Developed from the 1950s through the 1970s, plate tectonics is the modern version of continental drift, a theory first proposed by scientist Alfred Wegener in 1912. Wegener didn't have an explanation for how continents could move around the planet, but researchers do now. Plate tectonics is the unifying theory of geology, said Nicholas van der Elst, a seismologist at Columbia University's Lamont-Doherty Earth Observatory in Palisades, New York.
The driving force behind plate tectonics is convection in the mantle. Hot material near the Earth's core rises, and colder mantle rock sinks. "It's kind of like a pot boiling on a stove," Van der Elst said. The convection drive plates tectonics through a combination of pushing and spreading apart at mid-ocean ridges and pulling and sinking downward at subduction zones, researchers think. Scientists continue to study and debate the mechanisms that move the plates.
Mid-ocean ridges are gaps between tectonic plates that mantle the Earth like seams on a baseball. Hot magma wells up at the ridges, forming new ocean crust and shoving the plates apart. At subduction zones, two tectonic plates meet and one slides beneath the other back into the mantle, the layer underneath the crust. The cold, sinking plate pulls the crust behind it downward.
Many spectacular volcanoes are found along subduction zones, such as the "Ring of Fire" that surrounds the Pacific Ocean.
Plate boundaries
Subduction zones, or convergent margins, are one of the three types of plate boundaries. The others are divergent and transform margins.
At a divergent margin, two plates are spreading apart, as at seafloor-spreading ridges or continental rift zones such as the East Africa Rift.
Transform margins mark slip-sliding plates, such as California's San Andreas Fault, where the North America and Pacific plates grind past each other with a mostly horizontal motion.
With clues left behind in rocks and fossils, geoscientists can reconstruct the past history of Earth's continents. As the continents jostle around the Earth, they occasionally come together to form giant supercontinents, a single landmass. One of the earliest big supercontinents, called Rodinia. Its breakup is linked to a global glaciation called Snowball Earth. A more recent supercontinent called Pangaea.
Africa, South America, North America and Europe nestled closely together, leaving a characteristic pattern of fossils and rocks for geologists to decipher once Pangaea broke apart. The puzzle pieces left behind by Pangaea, from fossils to the matching shorelines along the Atlantic Ocean, provided the first hints that the Earth's continents move.
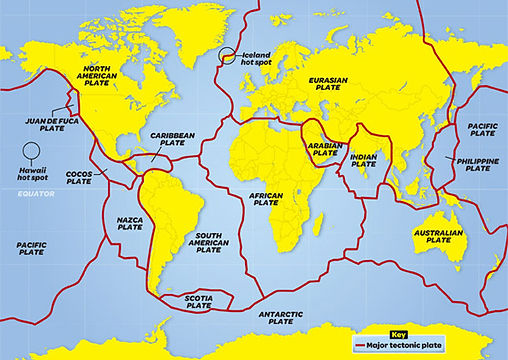
Pangea Theory or Expanding Earth
Pangaea or Pangea is a theory involving the concept that the earth's land mass at one point was gathered as a supercontinent that existed during the late Paleozoic and early Mesozoic eras. It assembled from earlier continental units approximately 300 million years ago, and it began to break apart about 175 million years ago. These time estimates are given based off what some scientist estimate the earth's age to be. Since time such great time is not truly observable we can only use certain limited resources to estimate a time for such events. As we understand all science to be evolving as we learn new facts these time frames should be considered as a theory rather than absolute. In contrast to the present Earth and its distribution of continental mass, much of Pangaea was in the southern hemisphere and surrounded by a super ocean, Panthalassa. Pangaea was the last supercontinent to have existed and the first to be reconstructed by geologists.
Plate Techtonics in action.
Plate tectonics is the theory that the outer rigid layer of the earth (the lithosphere) is divided into a couple of dozen "plates" that move around across the earth's surface relative to each other, like slabs of ice on a lake. Simplistically, the earth consists of the plates, and plate boundaries, those zones where the plates contact and interact.
NICKEL
The Earth is made out of many things. Deep inside Earth, near its center, lies Earth's core which is mostly made up of nickel and iron. Above the core is Earth's mantle, which is made up of rock containing silicon, iron, magnesium, aluminum, oxygen and other minerals.
Expanding Earth Theory
Different forms of the hypothesis
There are 3 forms of the expanding earth hypothesis.
-
Earth's mass has remained constant, and thus the gravitational pull at the surface has decreased over time.
-
Earth's mass has grown with the volume in such a way that the surface gravity has remained constant.
-
Earth's gravity at its surface has increased over time, in line with its hypothesized growing mass and volume.
The Expanding Earth hypothesis, first proposed by Robert Mantovani in 1889, attempts to explain continental drift, but without the actual continental drift.
This hypothesis was actually considered not unreasonable before the acceptance of plate tectonics, and there are still a small number of older respectable geologists who hold it plausible. It defied physics, but the geologists had been right and physics and chemistry wrong in the 19th century regarding the age of the Earth.
The hypothesis posits that the Earth is growing as a result of the expansion of the seafloor. According to its proponents, an "expanding Earth" model explains why the outlines of continents that were separated by continental drift do not quite match up (most people cite erosion as the major cause of this). If the Earth were, say, 20% smaller, it is claimed that South America and Africa would fit together like pieces in a jigsaw puzzle.
There are several versions of the hypothesis, from one that claims that the planet's mass has remained constant (and surface gravity has therefore decreased), to another that holds that the planet's mass has increased (where the extra mass came from is completely unaccounted for), to yet another that holds that the gravitational constant of the universe has changed.
Expansion with constant mass
In 1834, during the second voyage of HMS Beagle, Charles Darwin investigated stepped plains featuring raised beaches in Patagonia which indicated to him that a huge area of South America had been "uplifted to its present height by a succession of elevations which acted over the whole of this space with nearly an equal force." While his mentor Charles Lyell had suggested forces acting near the crust on smaller areas, Darwin hypothesized that uplift at this continental scale required "the gradual expansion of some central mass" [of the earth] "acting by intervals on the outer crust" with the "elevations being concentric with form of globe (or certainly nearly so)". In 1835 he extended this concept to include the Andes as part of a curved enlargement of the earth's crust due to "the action of one connected force". Not long afterwards, he moved on from this idea and proposed that as mountains rose, the ocean floor subsided, explaining the formation of coral reefs.
In 1889 and 1909 Roberto Mantovani published a hypothesis of Earth expansion and continental drift. He assumed that a closed continent covered the entire surface of a smaller Earth. Thermal expansion led to volcanic activity, which broke the land mass into smaller continents. Thermal expansion is the tendency of matter to change in shape, area, and/or volume in response to a change in temperature,[1]through heat transfer.These continents drifted away from each other because of further expansion at the rip-zones, where oceans currently lie. Although Alfred Wegener noticed some similarities to his own hypothesis of continental drift, he did not mention Earth expansion as the cause of drift in Mantovani's hypothesis.
A compromise between Earth-expansion and Earth-contraction is the "theory of thermal cycles" by Irish physicist John Joly. He assumed that heat flow from radioactive decayinside Earth surpasses the cooling of Earth's exterior. Together with British geologist Arthur Holmes, Joly proposed a hypothesis in which Earth loses its heat by cyclic periods of expansion. In their hypothesis, expansion led to cracks and joints in Earth's interior, that could fill with magma. This was followed by a cooling phase, where the magma would freeze and become solid rock again, causing Earth to shrink.
Problems with the hypothesis
The idea of an expanding Earth has several problems and very little evidence to back it up. For instance, in continental drift theory, measurements of the rate of plate movement (done by satellites and lasers) show the speed of movement. This movement, projected back, gives us the last time the continents were believed to have been together. These figures currently match up with geological strata and the fossil record.
Although some observations that lead to continental drift can also be explained by an expanding Earth, no conclusive evidence has been discovered that the Earth is expanding. The issue as to whether the Earth has increased in mass if gravity changed (and how) and if the Earth's density is altering are still issues that the expanding-Earth idea fails to address. How the concept fits in with the development of the solar system is also unknown, as presumably other planets and moons would also undergo expansion if it was possible; alternatively, one would need to explain why the Earth, and only the Earth, expands. If, at some point of the past, the radius of the Earth had been 50% of its current value, gravity force would be in the order of 39m/s², a value that would crush most humans, let alone bigger creatures like dinosaurs. Also, Earth density would work out at 44 kg/l, a value far exceeding that of the most dense materials on the planet. Modification of the gravity constants would have equally cathastrophic effects, related to the orbit of the Earth around the Sun.
The "theory" has not presented information to explain places where plates are crashing into each other - most notably, the creation of the Alps and the Himalayas due to the African and Indian plates, respectively, moving north and encountering the (relatively) immovable object of Eurasia.
Geophysical Global Cooling
The expanding Earth or growing Earth hypothesis asserts that the position and relative movement of continents is at least partially due to the volume of Earth increasing. Conversely, geophysical global cooling was the hypothesis that various features could be explained by Earth contracting.
Before the concept of plate tectonics, global cooling was a geophysical theory by James Dwight Dana, also referred to as the contracting earth theory. It suggested that theEarth had been in a molten state, and features such as mountains formed as it cooled and shrank. As the interior of the Earth cooled and shrank, the rigid crust would have to shrink and crumple. The crumpling could produce features such as mountain ranges.
Geophysics
Geophysics is a subject of natural science concerned with the physical processes and physical properties of the Earth and its surrounding space environment, and the use of quantitative methods for their analysis. The termgeophysics sometimes refers only to the geological applications: Earth's shape; its gravitational and magnetic fields; itsinternal structure and composition; its dynamics and their surface expression in plate tectonics, the generation ofmagmas, volcanism and rock formation. However, modern geophysics organizations use a broader definition that includes the water cycle including snow and ice; fluid dynamics of the oceans and the atmosphere; electricity and magnetism in the ionosphere and magnetosphere and solar-terrestrial relations; and analogous problems associated with the Moon and other planets.
Patagonia
Patagonia is a sparsely populated region located at the southern end of South America, shared by Argentina and Chile. The region comprises the southern section of the Andes mountains as well as the deserts, steppes and grasslands east of this southern portion of the Andes. Patagonia has two coasts; a western one towards the Pacific Ocean and an eastern one towards the Atlantic Ocean.
The Colorado and Barrancas rivers, which run from the Andes to the Atlantic, are commonly considered the northern limit of Argentine Patagonia. Tierra del Fuego, and sometimes the Falkland Islands are included as part of Patagonia. Most geographers and historians locate the northern limit of Chilean Patagonia at Reloncaví Estuary, To the South Chilean Patagonia is usually extended to the Straits of Magellan or prolonged to the Cape Horn.
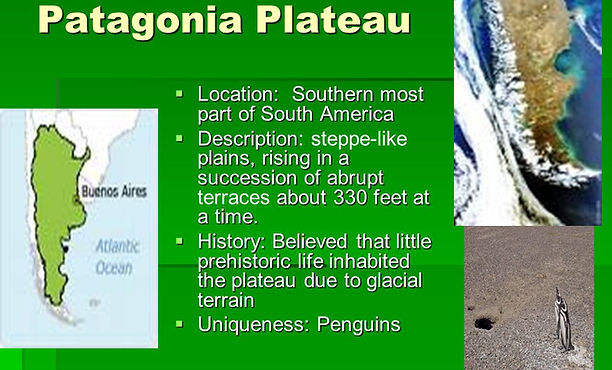
Earth has multiple layers. The ocean basins and the continents compose the crust, the outermost layer. Earth's crust is between three and 46 miles (five and 75 km) deep. The thickest parts are under the continents and the thinnest parts are under the oceans.
much of Earth's weather.
The mantle is the mostly-solid bulk of Earth's interior. The mantle lies between Earth's dense, super-heated core and its thin outer layer, the crust. The mantle is about 2,900 kilometers (1,802 miles) thick, and makes up a 84% of Earth's total volume. As Earth began to take shape, iron and nickel quickly separated from other rocks andminerals to form the core of the new planet. The molten material that surrounded the core was the early mantle. Over a period of time, the mantle cooled. Water trapped inside minerals erupted with lava, a process called “outgassing.” As more water was outgassed, the mantle solidified.
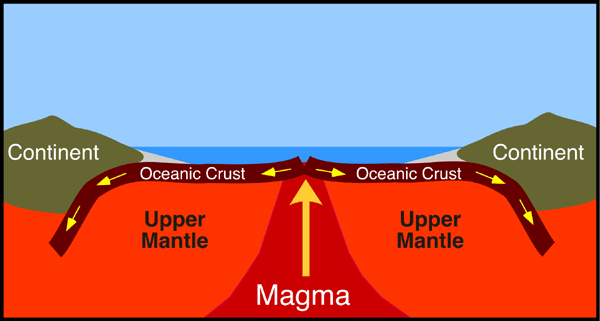
The rocks that make up Earth’s mantle are mostly silicates—a wide variety of compounds that share a silicon and oxygen structure. Common silicates found in the mantle include olivine, garnet, and pyroxene. The other major type of rock found in the mantle is magnesium oxide. Other mantle elements include iron, aluminum, calcium, sodium, and potassium.
The temperature of the mantle varies greatly, from 1000° Celsius (1832° Fahrenheit) near its boundary with the crust, to 3700° Celsius (6692° Fahrenheit) near its boundary with the core. In the mantle, heat and pressuregenerally increase with depth. The geothermal gradient is a measurement of this increase. In most places, the geothermal gradient is about 25° Celsius per kilometer of depth (1° Fahrenheit per 70 feet of depth).
The viscosity of the mantle also varies greatly. It is mostly solid rock, but more viscous at tectonic plate boundaries and mantle plumes. Mantle rocks there are soft and able to move plastically (over the course of millions of years) at great depth and pressure. The transfer of heat and material in the mantle helps determine the landscape of Earth. Activity in the mantle drives plate tectonics, contributing to volcanoes, seafloor spreading, earthquakes, and orogeny (mountain-building).
The mantle is divided into several layers: the upper mantle, the transition zone, the lower mantle, and D” (D double-prime), the strange region where the mantle meets the outer core.
Upper Mantle
The upper mantle extends from the crust to a depth of about 410 kilometers (255 miles). The upper mantle is mostly solid, but its more malleable regions contribute totectonic activity.
Two parts of the upper mantle are often recognized asdistinct regions in Earth’s interior: the lithosphere and the asthenosphere.
Lithosphere
The lithosphere is the solid, outer part of the Earth, extending to a depth of about 100 kilometers (62 miles). The lithosphere includes both the crust and the brittleupper portion of the mantle. The lithosphere is both the coolest and the most rigid of Earth’s layers.
The most well-known feature associated with Earth’s lithosphere is tectonic activity. Tectonic activity describes the interaction of the huge slabs of lithosphere calledtectonic plates. The lithosphere is divided into 15 major tectonic plates: the North American, Caribbean, South American, Scotia, Antarctic, Eurasian, Arabian, African, Indian, Philippine, Australian, Pacific, Juan de Fuca, Cocos, and Nazca.
The division in the lithosphere between the crust and the mantle is called the Mohorovicic discontinuity, or simply the Moho. The Moho does not exist at a uniform depth, because not all regions of Earth are equally balanced in isostatic equilibrium. Isostasy describes the physical, chemical, and mechanical differences that allow the crust to “float” on the sometimes more malleable mantle. The Moho is found at about 8 kilometers (5 miles) beneath the ocean and about 32 kilometers (20 miles) beneath continents.
Different types of rocks distinguish lithospheric crust and mantle. Lithospheric crust is characterized by gneiss (continental crust) and gabbro (oceanic crust). Below the Moho, the mantle is characterized by peridotite, a rock mostly made up of the minerals olivine and pyroxene.
Asthenosphere
The asthenosphere is the denser, weaker layer beneath the lithospheric mantle. It lies between about 100 kilometers (62 miles) and 410 kilometers (255 miles) beneath Earth’s surface. The temperature and pressure of the asthenosphere are so high that rocks soften and partly melt, becoming semi-molten.
The asthenosphere is much more ductile than either the lithosphere or lower mantle. Ductility measures a solid material’s ability to deform or stretch under stress. The asthenosphere is generally more viscous than the lithosphere, and the lithosphere-asthenosphere boundary (LAB) is the point where geologists and rheologists—scientists who study the flow of matter—mark the difference in ductility between the two layers of the upper mantle.
The very slow motion of lithospheric plates “floating” on the asthenosphere is the cause of plate tectonics, a process associated with continental drift, earthquakes, the formation of mountains, and volcanoes. In fact, the lava that erupts from volcanic fissures is actually the asthenosphere itself, melted into magma.
Of course, tectonic plates are not really floating, because the asthenosphere is not liquid. Tectonic plates are only unstable at their boundaries and hot spots.
Transition Zone
From about 410 kilometers (255 miles) to 660 kilometers (410 miles) beneath Earth’s surface, rocks undergo radical transformations. This is the mantle’s transition zone. In the transition zone, rocks do not melt or disintegrate. Instead, their crystalline structure changes in important ways. Rocks become much, much more dense. The transition zone prevents large exchanges of material between the upper and lower mantle. Some geologists think that the increased density of rocks in the transition zone prevents subducted slabs from the lithosphere from falling further into the mantle. These huge pieces of tectonic plates stall in the transition zone for millions of years before mixing with other mantle rock and eventually returning to the upper mantle as part of the asthenosphere, erupting as lava, becoming part of the lithosphere, or emerging as new oceanic crust at sites of seafloor spreading.
Some geologists and rheologists, however, think subducted slabs can slip beneath the transition zone to the lower mantle. Other evidence suggests that the transition layer is permeable, and the upper and lower mantle exchange some amount of material.
Water
Perhaps the most important aspect of the mantle’s transition zone is its abundance of water. Crystals in the transition zone hold as much water as all the oceans on Earth’s surface. Water in the transition zone is not “water” as we know it. It is not liquid, vapor, solid, or even plasma. Instead, water exists as hydroxide. Hydroxide is an ion of hydrogen and oxygen with a negative charge. In the transition zone, hydroxide ions are trapped in the crystalline structure of rocks such as ringwoodite and wadsleyite. These minerals are formed from olivine at very high temperatures and pressure.
Near the bottom of the transition zone, increasing temperature and pressure transform ringwoodite and wadsleyite. Their crystal structures are broken and hydroxide escapes as “melt.” Melt particles flow upwards, toward minerals that can hold water. This allows the transition zone to maintain a consistent reservoir of water.
Geologists and rheologists think that water entered the mantle from Earth’s surface during subduction. Subductionis the process in which a dense tectonic plate slips or melts beneath a more buoyant one. Most subduction happens as an oceanic plate slips beneath a less-dense plate. Along with the rocks and minerals of the lithosphere, tons of water and carbon are also transported to the mantle. Hydroxide and water are returned to the upper mantle, crust, and even atmosphere through mantle convection, volcanic eruptions, and seafloor spreading.
Lower Mantle
The lower mantle extends from about 660 kilometers (410 miles) to about 2,700 kilometers (1,678 miles) beneath Earth’s surface. The lower mantle is hotter and denser than the upper mantle and transition zone. The lower mantle is much less ductile than the upper mantle and transition zone. Although heat usuallycorresponds to softening rocks, intense pressure keeps the lower mantle solid. Geologists do not agree about the structure of the lower mantle. Some geologists think that subducted slabs of lithosphere have settled there. Other geologists think that the lower mantle is entirely unmoving and does not even transfer heat by convection.
D Double-Prime (D’’)
Beneath the lower mantle is a shallow region called D'', or “d double-prime.” In some areas, D’’ is a nearly razor-thin boundary with the outer core. In other areas, D’’ has thick accumulations of iron and silicates. In still other areas, geologists and seismologists have detected areas of huge melt.
The unpredictable movement of materials in D’’ isinfluenced by the lower mantle and outer core. The iron of the outer core influences the formation of a diapir, adome-shaped geologic feature (igneous intrusion) where more fluid material is forced into brittle overlying rock. The iron diapir emits heat and may release a huge, bulging pulse of either material or energy—just like a Lava Lamp. This energy blooms upward, transferring heat to the lower mantle and transition zone, and maybe even erupting as a mantle plume.
At the base of the mantle, about 2,900 kilometers (1,802 miles) below the surface, is the core-mantle boundary, or CMB. This point, called the Gutenberg discontinuity, marks the end of the mantle and the beginning of Earth’s liquid outer core.
Exploring the Mantle
The mantle has never been directly explored. Even the most sophisticated drilling equipment has not reached beyond the crust.
Drilling all the way down to the Moho (the division between the Earth's crust and mantle) is an important scientific milestone, but despite decades of effort, nobody has yet succeeded. In 2005, scientists with the Integrated Ocean Drilling Project drilled 1,416 meters (4,644 feet) below the North Atlantic seafloor and claimed to have come within just 305 meters (1,000 feet) of the Moho.
Xenoliths
Many geologists study the mantle by analyzing xenoliths. Xenoliths are a type of intrusion—a rock trapped inside another rock.
The xenoliths that provide the most information about the mantle are diamonds. Diamonds form under very unique conditions: in the upper mantle, at least 150 kilometers (93 miles) beneath the surface. Above depth and pressure, the carbon crystallizes as graphite, not diamond. Diamonds are brought to the surface in explosive volcanic eruptions, forming “diamond pipes” of rocks called kimberlites and lamprolites.
The diamonds themselves are of less interest to geologists than the xenoliths some contain. These intrusions are minerals from the mantle, trapped inside the rock-hard diamond. Diamond intrusions have allowed scientists to glimpse as far as 700 kilometers (435 miles) beneath Earth’s surface—the lower mantle.
Xenolith studies have revealed that rocks in the deep mantle are most likely 3-billion-year old slabs of subducted seafloor. The diamond intrusions include water, oceansediments, and even carbon.
Mantle Maps
Cutting-edge technology has allowed modern geologists and seismologists to produce mantle maps. Most mantle maps display seismic velocities, revealing patterns deep below Earth’s surface.
Geoscientists hope that sophisticated mantle maps can plot the body waves of as many as 6,000 earthquakes withmagnitudes of at least 5.5. These mantle maps may be able to identify ancient slabs of subducted material and the precise position and movement of tectonic plates. Many geologists think mantle maps may even provide evidence for mantle plumes and their structure.
Seismic Waves
Most mantle studies are conducted by measuring the spread of shock waves from earthquakes, called seismic waves. The seismic waves measured in mantle studies are called body waves, because these waves travel through the body of the Earth. The velocity of body waves differs with density, temperature, and type of rock.
There are two types of body waves: primary waves, or P-waves, and secondary waves, or S-waves. P-waves, also called pressure waves, are formed by compressions. Sound waves are P-waves—seismic P-waves are just far too low a frequency for people to hear. S-waves, also called shear waves, measure motion perpendicular to the energy transfer. S-waves are unable to transmit through fluids or gases.
Instruments placed around the world measure these waves as they arrive at different points on the Earth’s surface after an earthquake. P-waves (primary waves) usually arrive first, while s-waves arrive soon after. Both body waves “reflect” off different types of rocks in different ways. This allows seismologists to identify different rocks present in Earth’s crust and mantle far beneath the surface. Seismic reflections, for instance, are used to identify hidden oil deposits deep below the surface.
Sudden, predictable changes in the velocities of body waves are called “seismic discontinuities.” The Moho is a discontinuity marking the boundary of the crust and upper mantle. The so-called “410-kilometer discontinuity” marks the boundary of the transition zone.
The Gutenberg discontinuity is more popularly known as the core-mantle boundary (CMB). At the CMB, S-waves, which can’t continue in liquid, suddenly disappear, and P-waves are strongly refracted, or bent. This alerts seismologists that the solid and molten structure of the mantle has given way to the fiery liquid of the outer core.
Mantle Convection
Mantle convection describes the movement of the mantle as it transfers heat from the white-hot core to the brittle lithosphere. The mantle is heated from below, cooled from above, and its overall temperature decreases over long periods of time. All these elements contribute to mantle convection.
Convection currents transfer hot, buoyant magma to the lithosphere at plate boundaries and hot spots. Convection currents also transfer denser, cooler material from the crust to Earth’s interior through the process of subduction.
Earth's heat budget, which measures the flow of thermalenergy from the core to the atmosphere, is dominated by mantle convection. Earth’s heat budget drives most geologic processes on Earth, although its energy output isdwarfed by solar radiation at the surface.
Geologists debate whether mantle convection is “whole” or “layered.” Whole-mantle convection describes a long, long recycling process involving the upper mantle, transition zone, lower mantle, and even D’’. In this model, the mantle convects in a single process. A subducted slab of lithosphere may slowly slip into the upper mantle and fall to the transition zone due to its relative density and coolness. Over millions of years, it may sink further into the lower mantle. Convection currents may then transport the hot, buoyant material in D’’ back through the other layers of the mantle. Some of that material may even emerge as lithosphere again, as it is spilled onto the crust through volcanic eruptions or seafloor spreading.
Layered-mantle convection describes two processes. Plumes of superheated mantle material may bubble up from the lower mantle and heat a region in the transition zone before falling back. Above the transition zone, convection may be influenced by heat transferred from the lower mantle as well as discrete convection currents in the upper mantle driven by subduction and seafloor spreading. Mantle plumes emanating from the upper mantle may gush up through the lithosphere as hot spots.
Mantle Plumes
A mantle plume is an upwelling of superheated rock from the mantle. Mantle plumes are the likely cause of “hot spots,” volcanic regions not created by plate tectonics. As a mantle plume reaches the upper mantle, it melts into a diapir. This molten material heats the asthenosphere and lithosphere, triggering volcanic eruptions. These volcanic eruptions make a minor contribution to heat loss from Earth’s interior, although tectonic activity at plate boundaries is the leading cause of such heat loss.
The Hawaiian hot spot, in the middle of the North Pacific Ocean, sits above a likely mantle plume. As the Pacific plate moves in a generally northwestern motion, the Hawaiian hot spot remains relatively fixed. Geologists think this has allowed the Hawaiian hot spot to create a series of volcanoes, from the 85-million-year-old Meiji Seamount near Russia’s Kamchatka Peninsula, to the Loihi Seamount, a submarine volcano southeast of the “Big Island” of Hawaii. Loihi, a mere 400,000 years old, will eventually become the newest Hawaiian island.
Geologists have identified two so-called “superplumes.” These superplumes, or large low shear velocity provinces (LLSVPs), have their origins in the melt material of D’’. The Pacific LLSVP influences geology throughout most of the southern Pacific Ocean (including the Hawaiian hot spot). The African LLSVP influences the geology throughout most of southern and western Africa.
Geologists think mantle plumes may be influenced by many different factors. Some may pulse, while others may be heated continually. Some may have a single diapir, while others may have multiple “stems.” Some mantle plumes may arise in the middle of a tectonic plate, while others may be “captured” by seafloor spreading zones.
Some geologists have identified more than a thousand mantle plumes. Some geologists think mantle plumes don’t exist at all. Until tools and technology allow geologists to more thoroughly explore the mantle, the debate will continue.
The rocky surface layer of Earth, called the crust, is made up of mostly oxygen, silicon, aluminum, iron, calcium, sodium, potassium and magnesium. Earth's surface is mainly covered with liquid water and its atmosphere is is mainly nitrogen and oxygen, with smaller amounts of carbon dioxide, water vapor and other gases.
Earth is unique among the known planets: it has an abundance of water. Other worlds — including a few moons — have atmospheres, ice, and even oceans, but only Earth has the right combination to sustain life.
Earth's oceans cover about 70 percent of the planet's surface with an average depth of 2.5 miles (4 kilometers). Fresh water exists in liquid form in lakes and rivers and as water vapor in the atmosphere, which causes much of Earth's weather.
Crust
Earth's crust is made up of several elements: iron, 32 percent; oxygen, 30 percent; silicon, 15 percent; magnesium, 14 percent; sulfur, 3 percent; nickel, 2 percent; and trace amounts of calcium, aluminum and other elements.
The crust is divided into huge plates that float on the mantle, the next layer. The plates are constantly in motion; they move at about the same rate as fingernails grow. Earthquakes occur when these plates grind against each other. Mountains form when the plates collide and deep trenches form when one plate slides under another plate. Plate tectonics is the theory explaining the motion of these plates.
Mantle
The mantle under the crust is about 1,800 miles deep (2,890 km). It is composed mostly of silicate rocks called minerals rich in magnesium and iron. Intense heat causes the rocks to rise. They then cool and sink back down to the core. This convection — like a lava lamp — is believed to be what causes the tectonic plates to move. When the mantle pushes through the crust, volcanoes erupt.
Core
At the center of the Earth is the core, which has two parts. The solid, inner core of iron has a radius of about 760 miles (about 1,220 km). It is surrounded by a liquid, outer core composed of a nickel-iron alloy. It is about 1,355 miles (2,180 km) thick. The inner core spins at a different speed than the rest of the planet. This is thought to cause Earth's magnetic field. When charged particles from the solar wind collide with air molecules above Earth's magnetic poles, it causes the air molecules to glow, causing the auroras — the northern and southern lights.
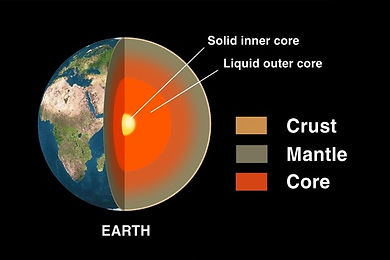
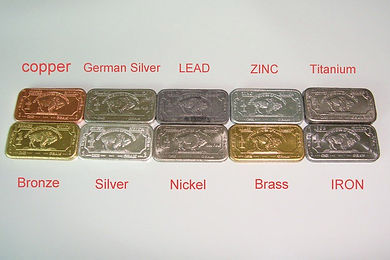